Abstract
Although messenger RNA (mRNA) has proved effective as a vaccine, its potential as a general therapeutic modality is limited by its instability and low translation capacity. To increase the duration and level of protein expression from mRNA, we designed and synthesized topologically and chemically modified mRNAs with multiple synthetic poly(A) tails. Here we demonstrate that the optimized multitailed mRNA yielded ~4.7–19.5-fold higher luminescence signals than the control mRNA from 24 to 72 h post transfection in cellulo and 14 days detectable signal versus <7 days signal from the control in vivo. We further achieve efficient multiplexed genome editing of the clinically relevant genes Pcsk9 and Angptl3 in mouse liver at a minimal mRNA dosage. Taken together, these results provide a generalizable approach to synthesize capped branched mRNA with markedly enhanced translation capacity.
This is a preview of subscription content, access via your institution
Access options
Access Nature and 54 other Nature Portfolio journals
Get Nature+, our best-value online-access subscription
$29.99 / 30 days
cancel any time
Subscribe to this journal
Receive 12 print issues and online access
$209.00 per year
only $17.42 per issue
Rent or buy this article
Prices vary by article type
from$1.95
to$39.95
Prices may be subject to local taxes which are calculated during checkout
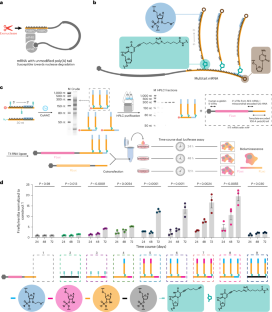
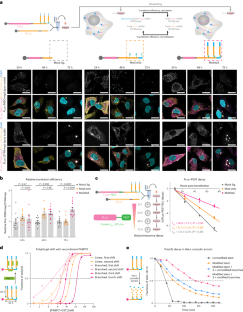
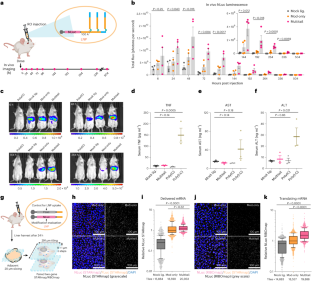

Data availability
NGS data were deposited to the NCBI Sequence Read Archive database under the accession code PRJNA107297178. All data supporting the findings of the presented study are listed in the article and Supplementary Information is available upon reasonable request.
References
-
Sahin, U., Karikó, K. & Türeci, Ö. mRNA-based therapeutics—developing a new class of drugs. Nat. Rev. Drug Discov. 13, 759–780 (2014).
-
Weng, Y. et al. The challenge and prospect of mRNA therapeutics landscape. Biotechnol. Adv. 40, 107534 (2020).
-
Rohner, E., Yang, R., Foo, K. S., Goedel, A. & Chien, K. R. Unlocking the promise of mRNA therapeutics. Nat. Biotechnol. 40, 1586–1600 (2022).
-
Baden, L. R. et al. Efficacy and safety of the mRNA-1273 SARS-CoV-2 vaccine. N. Engl. J. Med. 384, 403–416 (2021).
-
Walsh, E. E. et al. Safety and immunogenicity of two RNA-based COVID-19 vaccine candidates. N. Engl. J. Med. 383, 2439–2450 (2020)
-
Collén, A. et al. VEGFA mRNA for regenerative treatment of heart failure. Nat. Rev. Drug Discov. 21, 79–80 (2022).
-
Mullard, A. mRNA-based drug approaches phase I milestone. Nat. Rev. Drug Discov. 15, 595 (2016).
-
A study of VERVE-101 in patients with familial hypercholesterolemia and cardiovascular disease. Clinicaltrials.gov https://ift.tt/mJnSyCs (2023).
-
Rybakova, Y. et al. mRNA delivery for therapeutic anti-HER2 antibody expression in vivo. Mol. Ther. 27, 1415–1423 (2019).
-
Gillmore, J. D. et al. CRISPR–Cas9 in vivo gene editing for transthyretin amyloidosis. N. Engl. J. Med. 385, 493–502 (2021).
-
Ramaswamy, S. et al. Systemic delivery of factor IX messenger RNA for protein replacement therapy. Proc. Natl Acad. Sci. USA 114, E1941–E1950 (2017).
-
Jiang, L. et al. Dual mRNA therapy restores metabolic function in long-term studies in mice with propionic acidemia. Nat. Commun. 11, 5339 (2020).
-
Karikó, K. et al. Incorporation of pseudouridine into mRNA yields superior nonimmunogenic vector with increased translational capacity and biological stability. Mol. Ther. 16, 1833–1840 (2008).
-
Karikó, K., Buckstein, M., Ni, H. & Weissman, D. Suppression of RNA recognition by Toll-like receptors: the impact of nucleoside modification and the evolutionary origin of RNA. Immunity 23, 165–175 (2005).
-
Kormann, M. S. D. et al. Expression of therapeutic proteins after delivery of chemically modified mRNA in mice. Nat. Biotechnol. 29, 154–157 (2011).
-
Leppek, K. et al. Combinatorial optimization of mRNA structure, stability, and translation for RNA-based therapeutics. Nat. Commun. 13, 1536 (2022).
-
Asrani, K. H. et al. Optimization of mRNA untranslated regions for improved expression of therapeutic mRNA. RNA Biol. 15, 756–762 (2018).
-
Wesselhoeft, R. A., Kowalski, P. S. & Anderson, D. G. Engineering circular RNA for potent and stable translation in eukaryotic cells. Nat. Commun. 9, 2629 (2018).
-
Chen, R. et al. Engineering circular RNA for enhanced protein production. Nat. Biotechnol. https://ift.tt/CmVupin (2022).
-
Schlake, T., Thess, A., Thran, M. & Jordan, I. mRNA as novel technology for passive immunotherapy. Cell. Mol. Life Sci. 76, 301–328 (2019).
-
Thess, A. et al. Sequence-engineered mRNA without chemical nucleoside modifications enables an effective protein therapy in large animals. Mol. Ther. 23, 1456–1464 (2015).
-
Koch, A., Aguilera, L., Morisaki, T., Munsky, B. & Stasevich, T. J. Quantifying the dynamics of IRES and cap translation with single-molecule resolution in live cells. Nat. Struct. Mol. Biol. 27, 1095–1104 (2020).
-
Bloom, K., van den Berg, F. & Arbuthnot, P. Self-amplifying RNA vaccines for infectious diseases. Gene Ther. 28, 117–129 (2021).
-
Sonenberg, N. & Hinnebusch, A. G. Regulation of translation initiation in eukaryotes: mechanisms and biological targets. Cell 136, 731–745 (2009).
-
Kahvejian, A., Roy, G. & Sonenberg, N. The mRNA closed-loop model: the function of PABP and PABP-interacting proteins in mRNA translation. Cold Spring Harb. Symp. Quant. Biol. 66, 293–300 (2001).
-
Hinnebusch, A. G. The scanning mechanism of eukaryotic translation initiation. Annu. Rev. Biochem. 83, 779–812 (2014).
-
Eisen, T. J. et al. The dynamics of cytoplasmic mRNA metabolism. Mol. Cell 77, 786–799.e10 (2020).
-
Wang, Z., Day, N., Trifillis, P. & Kiledjian, M. An mRNA stability complex functions with poly(A)-binding protein to stabilize mRNA in vitro. Mol. Cell. Biol. 19, 4552–4560 (1999).
-
Mangus, D. A., Evans, M. C. & Jacobson, A. Poly(A)-binding proteins: multifunctional scaffolds for the post-transcriptional control of gene expression. Genome Biol. 4, 223 (2003).
-
Bernstein, P., Peltz, S. W. & Ross, J. The poly(A)-poly(A)-binding protein complex is a major determinant of mRNA stability in vitro. Mol. Cell. Biol. 9, 659–670 (1989).
-
Aditham, A. et al. Chemically modified mocRNAs for highly efficient protein expression in mammalian cells. ACS Chem. Biol. 17, 3352–3366 (2022).
-
Sawazaki, R. et al. Characterization of the multimeric structure of poly(A)-binding protein on a poly(A) tail. Sci. Rep. 8, 1455 (2018).
-
Kühn, U. & Pieler, T. Xenopus poly(A) binding protein: functional domains in RNA binding and protein-protein interaction. J. Mol. Biol. 256, 20–30 (1996).
-
Coombes, C. E. & Boeke, J. D. An evaluation of detection methods for large lariat RNAs. RNA 11, 323–331 (2005).
-
Katolik, A. et al. Regiospecific solid-phase synthesis of branched oligoribonucleotides that mimic intronic lariat RNA intermediates. J. Org. Chem. 79, 963–975 (2014).
-
Escorihuela, J. et al. Direct covalent attachment of DNA microarrays by rapid thiol-ene “click” chemistry. Bioconjug. Chem. 25, 618–627 (2014).
-
Greenberg, M. M. Attachment of reporter and conjugate groups to the 3′ termini of oligonucleotides. Curr. Protoc. Nucleic Acid Chem. https://ift.tt/pQ4X1Ed (2001).
-
El-Sagheer, A. H. & Brown, T. Single tube gene synthesis by phosphoramidate chemical ligation. Chem. Commun. 53, 10700–10702 (2017).
-
Kalinowski, M. et al. Phosphoramidate ligation of oligonucleotides in nanoscale structures. ChemBioChem 17, 1150–1155 (2016).
-
Ehret, F., Zhou, C. Y., Alexander, S. C., Zhang, D. & Devaraj, N. K. Site-specific covalent conjugation of modified mRNA by tRNA guanine transglycosylase. Mol. Pharm. 15, 737–742 (2018).
-
Zhang, D. et al. Site-specific and enzymatic cross-linking of sgRNA enables wavelength-selectable photoactivated control of CRISPR gene editing. J. Am. Chem. Soc. 144, 4487–4495 (2022).
-
Fantoni, N. Z., El-Sagheer, A. H. & Brown, T. A hitchhiker’s guide to click-chemistry with nucleic acids. Chem. Rev. 121, 7122–7154 (2021).
-
Warminski, M., Kowalska, J. & Jemielity, J. Solid-phase synthesis of RNA 5′-azides and their application for labeling, ligation, and cyclization via click chemistry. Curr. Protoc. Nucleic Acid Chem. 82, e112 (2020).
-
Kühn, U. & Wahle, E. Structure and function of poly(A) binding proteins. Biochim. Biophys. Acta 1678, 67–84 (2004).
-
Deo, R. C., Bonanno, J. B., Sonenberg, N. & Burley, S. K. Recognition of polyadenylate RNA by the poly(A)-binding protein. Cell 98, 835–845 (1999).
-
Vogel, A. B. et al. BNT162b vaccines protect rhesus macaques from SARS-CoV-2. Nature 592, 283–289 (2021).
-
Gilleron, J. et al. Image-based analysis of lipid nanoparticle-mediated siRNA delivery, intracellular trafficking and endosomal escape. Nat. Biotechnol. 31, 638–646 (2013).
-
Wang, X. et al. Three-dimensional intact-tissue sequencing of single-cell transcriptional states. Science 361, eaat5691 (2018).
-
Zeng, H. et al. Integrative in situ mapping of single-cell transcriptional states and tissue histopathology in a mouse model of Alzheimer’s disease. Nat. Neurosci. 26, 430–446 (2023).
-
Zeng, H. et al. Spatially resolved single-cell translatomics at molecular resolution. Science 380, eadd3067 (2023).
-
Xiang, K. & Bartel, D. P. The molecular basis of coupling between poly(A)-tail length and translational efficiency. eLife 10, e66493 (2021).
-
Li, X. et al. Generation of destabilized green fluorescent protein as a transcription reporter. J. Biol. Chem. 273, 34970–34975 (1998).
-
Nicholson-Shaw, A. L., Kofman, E. R., Yeo, G. W. & Pasquinelli, A. E. Nuclear and cytoplasmic poly(A) binding proteins (PABPs) favor distinct transcripts and isoforms. Nucleic Acids Res. 50, 4685–4702 (2022).
-
Perzanowska, O., Smietanski, M., Jemielity, J. & Kowalska, J. Chemically modified poly(A) analogs targeting PABP: structure activity relationship and translation inhibitory properties. Chemistry 28, e202201115 (2022).
-
Görlach, M., Burd, C. G. & Dreyfuss, G. The mRNA poly(A)-binding protein: localization, abundance, and RNA-binding specificity. Exp. Cell. Res. 211, 400–407 (1994).
-
Schäfer, I. B. et al. Molecular basis for poly(A) RNP architecture and recognition by the Pan2–Pan3 deadenylase. Cell 177, 1619–1631.e21 (2019).
-
Webster, M. W. et al. mRNA deadenylation is coupled to translation rates by the differential activities of Ccr4–Not nucleases. Mol. Cell 70, 1089–1100.e8 (2018).
-
Dehlin, E., Wormington, M., Körner, C. G. & Wahle, E. Cap-dependent deadenylation of mRNA. EMBO J. 19, 1079–1086 (2000).
-
Ruud, K. A., Kuhlow, C., Goss, D. J. & Browning, K. S. Identification and characterization of a novel cap-binding protein from Arabidopsis thaliana. J. Biol. Chem. 273, 10325–10330 (1998).
-
Shestakova, E. D., Smirnova, V. V., Shatsky, I. N. & Terenin, I. M. Specific mechanisms of translation initiation in higher eukaryotes: the eIF4G2 story. RNA 29, 282–299 (2023).
-
Ho, J. J. D. et al. Systemic reprogramming of translation efficiencies on oxygen stimulus. Cell Rep. 14, 1293–1300 (2016).
-
Moerke, N. J. et al. Small-molecule inhibition of the interaction between the translation initiation factors eIF4E and eIF4G. Cell 128, 257–267 (2007).
-
Jang, D.-I. et al. The role of tumor necrosis factor alpha (TNF-α) in autoimmune disease and current TNF-α inhibitors in therapeutics. Int. J. Mol. Sci. 22, 2719 (2021).
-
Giannini, E. G., Testa, R. & Savarino, V. Liver enzyme alteration: a guide for clinicians. CMAJ 172, 367–379 (2005).
-
Kumar, A., Zhang, J. & Yu, F.-S. X. Toll-like receptor 3 agonist poly(I:C)-induced antiviral response in human corneal epithelial cells. Immunology 117, 11–21 (2006).
-
Okahira, S. et al. Interferon-beta induction through Toll-like receptor 3 depends on double-stranded RNA structure. DNA Cell Biol. 24, 614–623 (2005).
-
Rothgangl, T. et al. In vivo adenine base editing of PCSK9 in macaques reduces LDL cholesterol levels. Nat. Biotechnol. 39, 949–957 (2021).
-
Robson, A. Three different therapies to target PCSK9. Nat. Rev. Cardiol. 18, 541 (2021).
-
Nelson, C. E. et al. Long-term evaluation of AAV–CRISPR genome editing for Duchenne muscular dystrophy. Nat. Med. 25, 427–432 (2019).
-
Musunuru, K. et al. In vivo CRISPR base editing of PCSK9 durably lowers cholesterol in primates. Nature 593, 429–434 (2021).
-
Qiu, M. et al. Lipid nanoparticle-mediated codelivery of Cas9 mRNA and single-guide RNA achieves liver-specific in vivo genome editing of Angptl3. Proc. Natl Acad. Sci. USA 118, e2020401118 (2021).
-
Zhou, J. et al. Dual sgRNAs facilitate CRISPR/Cas9-mediated mouse genome targeting. FEBS J. 281, 1717–1725 (2014).
-
Park, J. et al. Short poly(A) tails are protected from deadenylation by the LARP1–PABP complex. Nat. Struct. Mol. Biol. https://ift.tt/r7nAQMh (2023).
-
Arevalo, C. P. et al. A multivalent nucleoside-modified mRNA vaccine against all known influenza virus subtypes. Science 378, 899–904 (2022).
-
Foy, S. P. et al. Non-viral precision T cell receptor replacement for personalized cell therapy. Nature 615, 687–69 (2023).
-
Dong, Y. et al. DNA functional materials assembled from branched DNA: design, synthesis, and applications. Chem. Rev. 120, 9420–9481 (2020).
-
Horn, T., Chang, C. A. & Urdea, M. S. Chemical synthesis and characterization of branched oligodeoxyribonucleotides (bDNA) for use as signal amplifiers in nucleic acid quantification assays. Nucleic Acids Res. 25, 4842–4849 (1997).
-
Chen, H. et al. Branched, chemically modified poly(A) tails enhance the translation capacity of mRNA. Sequence Read Archive https://ift.tt/7EYbp3H (2024).
-
Anzalone, A. V. et al. Programmable deletion, replacement, integration and inversion of large DNA sequences with twin prime editing. Nat. Biotechnol. 40, 731–740 (2022).
Acknowledgements
We thank the MIT Department of Chemistry Instrument Facility for providing instrument access and N. Ye (MIT) for assistance on protein purification. We thank H. Zeng (Broad Institute) for his help in designing the STARmap/RIBOmap probes. We also thank other members of X.W.’s laboratory for helpful discussion throughout the project. X.W. acknowledges the support from the Searle Scholars Program, Thomas D. and Virginia W. Cabot Professorship, E. Scolnick Professorship, Ono Pharma Breakthrough Science Initiative Award, Merkin Institute Fellowship, and NIH DP2 New Innovator Award (1DP2GM146245-01). A.H. is a National Science Foundation Graduate Research Fellow. A.H. and D.R.L. were supported by NIH U01AI142756, R35GM118062, RM1HG009490 and the Howard Hughes Medical Institute (HHMI). This article is subject to HHMI’s Open Access to Publications policy. HHMI lab heads have previously granted a nonexclusive CC BY 4.0 license to the public and a sublicensable license to HHMI in their research articles. Pursuant to those licenses, the author-accepted manuscript of this article can be made freely available under a CC BY 4.0 license immediately upon publication.
Author information
Authors and Affiliations
Contributions
X.W. conceived the project. H.C. and X.W. designed experiments. H.C., D.L., J.G., A.A., Y.Z., J.T., J.R., A.H., F.K. and M.W. performed the experiments. H.C., J.G., S.L., A.H. and J.H. performed data analysis. X.W. supervised the work. H.C. and X.W. wrote the paper with input from all authors.
Corresponding author
Ethics declarations
Competing interests
X.W., H.C., A.A. and J.G. are inventors on patent applications related to branched RNA. X.W. is a scientific cofounder, consultant and equity holder of Stellaromics and Convergence Therapeutics. D.R.L. is a cofounder, consultant and equity holder of Beam Therapeutics, Prime Medicine, Pairwise Plants, Chroma Medicine, Exo Therapeutics and Nvelop Therapeutics. The other authors declare no competing interests.
Peer review
Peer review information
Nature Biotechnology thanks the anonymous reviewers for their contribution to the peer review of this work.
Additional information
Publisher’s note Springer Nature remains neutral with regard to jurisdictional claims in published maps and institutional affiliations.
Extended data
Extended Data Fig. 1 Screening of oligonucleotide crosslinking chemistry.
(a) Summary of oligonucleotide chemical conjugation methods. Screening was performed using 15-nt poly-deoxyadenosine model substrates at micromolar concentrations. Modification handles were incorporated through oligonucleotide solid phase synthesis, followed by amine-NHS labeling and HPLC purification. (b) Gel electrophoresis of crude thiol-ene/yne oligonucleotide conjugation of 15-nt model substrates containing only one conjugation handle. (c) Gel electrophoresis of crude CuAAC and IEDDA 30-nt oligonucleotides bearing three alkyne/trans-cyclooctene handles reacting with 30-nt azide/tetrazine modified oligo. (d) Preliminary dual luciferase assay using branched mRNA prepared from CuAAC product mixture without HPLC purification using different equivalents of branched oligos versus stem oligo. Branching oligos contained modifications on the last six bases with either natural (5′ to 3′) or reversed (3′ to 5′) directionality. Time-course assay was performed as outlined in Fig. 1. n = 3 independent transfections for each construct. Mean ± s.e.m. P values were calculated by ordinary two-sided one-way ANOVA (comparison of means across time points). Gels are representative of at least two experiments.
Source data
Extended Data Fig. 2 Synthesis and characterization of topologically augmented branched mRNA.
(a-c) Representative HPLC purification and gel electrophoresis characterizations of branched oligonucleotides (oligo) containing 1-3 branching poly(A) tails. Fractions containing the desired products (boxed) were pooled and isolated. (d) Schematics of preparation of firefly luciferase (FLuc) mRNA constructs lacking poly(A) tail before ligation. FLuc mRNA without template encoded poly(A) tail was ligated to a scramble (non-polyA) stem oligo with 3′ end modifications (six phosphorothioate/2MOE at 3′ and terminal dideoxycytidine modifications) and internal alkyne (5-octadiynyl deoxyuridine or OU), with or without conjugation to 5′ azide labeled scramble or poly(A) branches with 3′ end modifications (the same as the stem oligo). (e) RNase H characterization of branched mRNA-oligo conjugates with branched poly(A) or scramble tails. The branching topology was confirmed by further band shift on TBU gel. (f) Branched poly(A) sequence, rather than chemical modifications alone, conferred enhanced protein expression over time. Relative FLuc luminescence was normalized to the scramble OU only oligo ligated mRNA at indicated time points. n = 3 independent transfections for each construct. Mean ± s.e.m. P values were calculated by two-sided unpaired t-test (with Welch’s correction) against the scramble OU + scramble azide construct at corresponding time points. (g) RNase H characterization of branched mRNA-oligo conjugates with multiple ploy(A) tails. mRNA with full-length hemoglobin UTRs and template encoded 100A-tail was ligated to 0 (mock ligation), 30 A, 60 A, or oligos with one, two, or three branched poly(A) tails and characterized by RNase H assay. The branching topology was confirmed by further band shift on TBU gel. Gels are representative of at least two experiments.
Source data
Extended Data Fig. 3 Dissecting the effects of branched poly(A) tails on mRNA stability and translation efficiency.
(a) Representative STARmap/RIBOmap images were acquired under the same confocal imaging settings from three independent experiments for each condition. STARmap versus RIBOmap characterization of different modified Firefly luciferase (FLuc) mRNA constructs and STARmap characterization of internal control Renilla luciferase (RLuc) mRNA were performed as outlined in Fig. 2. DAPI (blue), nuclei; FLuc amplicons (magenta); RLuc amplicons (yellow). Colocalized FLuc/RLuc amplicons in STARmap (white dots) were lipid transfection vesicles and were excluded from downstream quantification. Scale bar = 100 µm. (b-c) Violin plots of single-cell quantification of FLuc STARmap/RIBOmap amplicons. P values were calculated by ordinary two-sided two-way ANOVA. (d) Decay kinetics of internal control RLuc luminescence outlined in Fig. 2c normalized to luminescence at 8 hrs post transfection. n = 3 independent transfections. P values were calculated by ordinary two-sided two-way ANOVA (with Geisser-Greenhouse correction, decay across time points).
Source data
Extended Data Fig. 4 Branched poly(A) tails bind PABPC1 protein.
(a) Electrophoretic mobility shift assay of three branched poly(A) oligo with varying concentrations of GST-tagged recominant human PABPC1 protein (PABPC1-GST). The stem oligo was labeled with Alexa Fluore 546 at 5′end. Both stem and branching poly(A)’s were 30 nt and modified with PS-2MOE at the last six bases and ddC at the 3′ end. (b) Gel shift assay of the same modified poly(A) oligo without branching. Gels are representative of at least two experiments.
Source data
Extended Data Fig. 5 Chemical and topological augmentation stabilized poly(A) tails against nuclease degradation in vitro.
(a-d) Evaluation of chemically and topologically modified poly(A) tails in CAF1-CCR4 deadenylation assay. Four different Alexa-546 labeled poly(A) oligos were subjected to deadenylation using recombinant CAF1/CCR4 protein complex over the course of 110 min: linear unmodified 60 A oligo (a), end modified (six PS-2MOE at 3′ and terminal dideoxycytidine, the same set of modifications for all other oligos) 30 A oligo (b), end modified 30 A stem oligo + unmodified 30 A branches (c), and end modified stabilized 30 A stem oligo + end modified 30 A branches (d). (e-h) Evaluation of chemically and topologically modified poly(A) tails in HeLa cell lysate. Alexa-546 labeled poly(A) oligos (the same four constructs used in a-d) were subjected to digestion in HeLa cytosolic lysate for 220 min: linear unmodified 60 A oligo (e), end modified 30 A oligo (f), end modified 30 A stem oligo + unmodified 30 A branches (g), and end modified 30 A stem oligo + end modified 30 A branches (h). Gels were representative of two experiments.
Source data
Extended Data Fig. 6 Multi-tailed mRNA depends on the canonical eIF4-eIF3 translation initiation mechanism.
(a) Timeline of the knockdown (KD) experiments. HeLa cells were treated with siRNA cocktails targeting the corresponding eIFs 48 hrs post siRNA transfection, cells were reseeded and transfected with mRNA followed by protein quantification after 6 hrs. Successful knockdowns were confirmed by western blots and RT-qPCR at 48 hrs. (b,c,e) KD experiments for eIF4E/eIF4G/eIF3D. (d) Comparison of multi-tailed mRNA to regular mRNA in 4EGI-1 treated in vitro translation assay using rabbit reticulocyte lysate (RRL). Luciferase expressions were normalized to the wild-type condition for each construct. Mean± s.e.m. n = 3. P values were calculated by two-sided unpaired t-test for intra-construct comparison (KD vs WT) and by two-sided one-way ANOVA for cross-construct comparison. Western blots were representative of two experiments.
Source data
Extended Data Fig. 7 Comparison of UTR optimized circRNA and multi-tailed mRNA using secreted NanoLuc reporter.
(a) Gel electrophoresis of circRNA encoding secreted Nanoluc (IL6-Nluc) generated through IVT, backsplicing, and enriched by RNase R treatment. Successful circularization was confirmed by RNase R resistance and slower mobility on TBU gel compared to corresponding intron-free linear RNA. (b) Comparison of UTR optimized circRNA and multi-tailed mRNA using IL6-NLuc 1 day after transfection with indicated amounts of RNA. Mock lig./mod-only/multi-tail mRNAs contained optimized UTRs, full m1ψ replacement and 100 A through IVT with mock ligation (mock lig.), ligated to modification-only oligo (mod-only), or modified multi-tailed oligo (multi-tail). CircRNAs were designed to contain optimized HRV IRES (with proximal loop Apt-eIF4G insertion) and 3′-PABP binding motif without addition of modified nucleotides. Cells were cultured in phenol red-free media. On each day, media was completely harvested and renewed with 150 μL fresh media and 5 μL of old media was diluted and used for luciferase assay. n = 3 independent transfections in each biological condition. Mean ± s.e.m. (c) IL6-Nluc signal over 14 days. n = 3 independent transfections in each biological condition. Mean ± s.e.m.
Source data
Extended Data Fig. 8 Paired STARmap/RIBOmap effectively characterize the quantity and translatability of mRNA constructs delivered by LNP to murine liver.
(a) Schematics for evaluating two-gene STARmap/RIBOmap experiments in different lobes of murine liver. 2:1 NLuc/FLuc mRNA were co-encapsulated in LNP and administered by retro-orbital (R.O.) injection. 6 hrs post mRNA injection, the mouse liver was harvested in five lobes (caudate, left/right medial and lateral lobes). Each lobe was sections into two adjacent 10-μm slices, with one slice profiled by NLuc STARmap and FLuc STARmap and the other slice profiled by NLuc RIBOmap and Fluc STARmap. Whole sections were profiled in 291×291 μm2 tiles. (b, c) Quantification of numbers of NLuc and FLuc amplicons in each tile. n = 3379/3199 tiles. Slopes (NLuc/FLuc) were calculated by linear regression (least-square, Q = 5% for outlier rejection).
Source data
Extended Data Fig. 9 Delivery of CRISPR editing using chemically and topologically modified mRNA.
(a) Comparison of Cas9 expression by western blot 48 hrs post transfection in HEK293T-uGFP cells. NLS, nuclear localization signal. Western blots were representative of two experiments. (b) FACS gating strategy for uGFP positive HEK293T cells. (c) Exemplary single-cell uGFP quantification for cells treated with unligated Cas9 mRNA only (Cas9-only) without sgRNAs, or with mock lig./mod-only/multi-tail Cas9 mRNA co-transfected with two sgRNAs. FACS were performed 72 hrs post mRNA transfection. n = 11845/10983/12334/12131 single cells. P value was calculated by two-sided one-way ANOVA. (d-h) Serum levels of Pcsk9 protein (d), Angptl3 protein (e), free cholesterol (f), total cholesterol (g), and triglyceride (h) over 4 weeks normalized to the Cas9-only group. Mean ± s.e.m. n = 4, biological replicates.
Source data
Supplementary information
Reporting Summary
Supplementary Table 1
Compiled sequences of genes/oligos/probes used in the study.
Source data
Source Data Figs. 1–4 and Extended Data Figs. 1–9
Compiled statistical source data for all figures presented.
Rights and permissions
Springer Nature or its licensor (e.g. a society or other partner) holds exclusive rights to this article under a publishing agreement with the author(s) or other rightsholder(s); author self-archiving of the accepted manuscript version of this article is solely governed by the terms of such publishing agreement and applicable law.
Reprints and permissions
About this article
Cite this article
Chen, H., Liu, D., Guo, J. et al. Branched chemically modified poly(A) tails enhance the translation capacity of mRNA. Nat Biotechnol (2024). https://ift.tt/yUZ2p9t
-
Received:
-
Accepted:
-
Published:
-
DOI: https://ift.tt/yUZ2p9t
No comments:
Post a Comment